1.IntroductionThe interaction of hemoglobin (Hb) with its natural environment, the red blood cell (RBC), is complex and encompasses such different processes as oxygen transport, export of nitric oxide bioactivity,1 Hb binding to the cell membrane,2 and conversion of Hb to inactive forms (aging).3 There is an obvious motivation to learn more about these processes because malfunction of Hb, or the red blood cell, gives rise to disease. Given the rich dynamics of the Hb-RBC interplay, development of new tools for studying RBCs is desirable. Raman spectroscopy, i.e., inelastic light scattering from vibrational modes, is an attractive optical technique because it provides direct access to the state of hemoglobin. The porphyrin groups in Hb absorb visible light and a resonance effect leads to a selective enhancement of the porphyrin vibrations in the Raman spectrum. Raman scattering from Hb provides information about the oxygenation state, as well as the oxidation and spin state of the heme irons.4 5 6 7 Most previous Raman investigations have been carried out on isolated and purified Hb. There have also been early studies of Hb within cells,4 8 in which Hb spectra did not differ compared to free Hb spectra. Recently, Raman spectroscopy of single cells, and a near-infrared Raman spectroscopy study of single optically trapped biological cells, was reported.9 10 11 12 Inspired by these studies, we decided to study single erythrocytes using Raman spectroscopy. The first reason is that individual cells might behave in a different way than the ensemble average. The second motivation is that the size and morphology of the RBCs are used as diagnostic indicators. Additional information on the spatial location of various Hb states inside the cell would be of considerable value. A practical problem in studies of individual cells is the choice of preparation method. Ideally, the cell should be immobilized without changing the intrinsic properties, while permitting variation of the external environment. In the first Raman spectroscopic investigation of single erythrocytes, the cells were immobilized by methanol fixation on a glass slide in air.9 Although Ong et al.; showed that this method allowed for successful screening for RBCs infected by a parasite, the cells were obviously not in an in-vivo environment. RBCs exposed to air might also be more susceptible to photo-induced chemical reactions, like photo-oxidation, even at low illumination power. Negative effects of laser irradiation on cells, e.g., on human lymphocytes and chromosomes, have been reported and it was found that the right choice of excitation wavelength is crucial to prevent cell degradation.13 Clearly, the recent reports by Wood, Tait, and McNaughton10 11 is promising in this respect, since they were able to assign Raman bands of Hb in both oxygenation states within single RBCs using 632.8-nm excitation, and no cell degradation was observed. The goal of this study was to investigate the feasibility of the Raman scattering technique applied to single human RBCs immobilized on polylysine-coated glass slides in appropriate buffer solutions. In particular, the biocompatibility of two glass surfaces, covered with polylysine of varying molecular weight, was compared by taking Raman spectra and global Raman images. In the latter case, the sample is globally illuminated with a defocused laser beam. Back-scattered light is collected within a narrow wave number interval (20 cm−1) and imaged on a CCD chip. By doing so, a global Raman image showing the distribution of a compound in the sample can be obtained.14 15 We found that both the nature of the glass coating and the laser irradiation had an important influence on the Raman spectra and global Raman images of RBCs and Hb. Using visible excitation (λ=514.5 nm), both fluorescence and Hb Raman scattering was measured. Furthermore, we could record series of Raman spectra during the oxygenation course from one immobilized erythrocyte. This demonstrates that Hb in RBCs remained functional over a long period of time. The method is therefore suitable for further investigations of Hb dynamics within single cells under various conditions, although extreme care has to be taken to avoid spurious effects caused by the measurement itself. A preliminary account of this work has been presented at a recent conference.16 2.Materials and MethodsExperiments were performed with a Renishaw Raman Imaging Microscope 2000 setup (see Fig. 1). Raman spectra and global Raman images were measured using a 60× water immersion objective, NA=0.9, in back-scattering geometry. The spectral resolution of the single spectrograph and of the bandpass filter was measured to be 6 and 20 cm−1, respectively. The Raman excitation source was an Ar + -laser (Spectraphysics Beam Lock 2060) tuned to 514.5 nm. Raman spectra were usually taken with a power of 0.7 mW onto the sample and the laser beam was focused to a diameter of ∼1 μm. A typical irradiance was thus ∼90 kW/cm2. Global Raman images were recorded with a defocused laser beam (30 μm) and a laser power of 3.2 mW onto the sample, which corresponds to an irradiance of ∼450 W/cm2. For global Raman imaging, a reference image from a Si sample was taken before each measurement to adjust for inhomogeneous sample illumination. Figure 1Schematic of the Raman spectrometer setup. The laser beam path is illustrated by arrows. The cells are viewed with a water-immersion objective, which allows for addition of sodium dithionite, or exchange of buffers, while recording spectra from the immobilized cell. Back-scattered Raman light is collected by the same objective and directed either to the grating spectrometer, or to a narrow bandpass filter, and finally detected by a CCD. ![]() Erythrocytes were immobilized on two kinds of poly-L-lysine covered glass surfaces, in the following termed surface A and surface B, and covered with a drop of Hepes buffer solution (pH 7.2, ∼200 μl). Surface A corresponds to commercial poly prep slides (SIGMA, coated with poly-L-lysine, molecular weight 150 000 to 300 000). Surface B corresponds to slides coated with poly-L-lysine hydrobromide (SIGMA Company, molecular weight between 70 000 to 150 000), prepared by the following procedure: commercial glass slides were cleaned by putting them in a solution consisting of five parts MilliQ-purified water and one part ammoniac (15). After warming up the solution to 80°C, one part of hydrogen peroxide (30, 4 ml) was added. The slides were boiled in the solution for 5 min and then carefully rinsed with water and dried under a nitrogen stream. The bioadhesive film was generated by immersing the slides in a 50 μg/ml polylysine/Hepes buffer solution (pH 7.2) for at least 2 h, after which the slides were rinsed with buffer. Human adult blood (5 μl), drawn from the fingertip of the healthy author, was directly diluted in 2 ml of the Hepes buffer. In the case of surface A, the solution was put directly on the slide and put onto the microscope table so that the adsorption of the RBCs could be followed directly in real time. In the case of surface B, 50 μl of the solution was put on the poly-L-lysine covered slide for 2 min, after which excess erythrocytes were washed away by rinsing the slide with Hepes buffer. The slide was finally mounted on the microscope table. The cells were kept in buffer at all times. Molecular oxygen could be removed from the buffer by adding ∼0.5-mg sodium dithionite to the 200-μl buffer droplet. The sample setup has the advantage that the surrounding buffer can be exchanged during the course of an experiment and that chemicals can be added to the buffer while keeping the same cell in the laser focus. The buffer also reduces thermal heating by the laser irradiation. The temperature rise at thermal equilibrium of the illuminated volume due to absorption of laser light can be roughly estimated from the equation where Pa is the power absorbed by the illuminated cell volume.17 In this model, the sample is assumed to be a homogeneous sphere immersed in an isotropic medium with thermal conductivity K. We assume that K cell ≅K substrate ≅K water =0.6 W/mK. The radius ra is that of a sphere with a volume equivalent to the illuminated volume, which is approximately given by the product of the cell height (∼2 μm) and the illuminated area (∼1 μm2). The absorbed power is given by Pa=σaP/A cell , where σa is the absorption cross section for a single cell, P is the laser power at the sample (∼0.7 mW), and A cell is the geometrical cross section of the cell (∼50 μm2). The absorption cross section was estimated to ∼0.875 μm2 at 514.5 nm, by measuring extinction spectra for a monolayer of erythrocytes using a Cary 500 spectrophotometer and subtracting a linear base line caused by elastic scattering. The resulting temperature rise is computed to ∼2.3°C, which implies that the actual cell temperature should be well below physiological conditions.3.Experimental Results and DiscussionTo investigate the possibility of photo-induced effects, we measured Raman spectra as a function of time at constant irradiance. The spectra in Fig. 2 show that both the Raman spectra and the fluorescence from single RBCs, immobilized to surface A and B in buffer, change on illumination. In particular, we observed that the Raman signal intensity decreased with time. A gradual decrease of the Raman signal has also been observed by Puppels et al.; in a Raman study on human lymphocytes and chromosomes,13 where the decrease was attributed to cellular degradation. However, the decrease of the Raman signal reported here occurred simultaneously with change of the Hb Raman spectra. This is illustrated in Fig. 2, where the ratio between the 1589 and the 1640 cm−1 peaks are plotted versus time. As a comparison, the same ratio for a typical crystalline spectrum of a met-Hb is included in the same graph. The similarity to the spectra taken after 540 s strongly indicate a change in hemoglobin state from oxy-Hb (2+) to the inactive met-Hb (3+), for which the oxygen is irreversible bound to the heme group.7 Figure 2Photo-induced conversion of Hb. The plots above (a) show series of spectra recorded from the two types of glass slides used. The integration time was 80 s/spectrum (at an interval of 3 s); the irradiance was 90 kW/cm2. For both surfaces an increase of the fluorescence background appears already after the first spectrum. By the end of the time series, part of the oxy-Hb had turned into the met-Hb state. The plots below (b) show how the Raman signal at 1640 cm−1 and the fluorescence background at 2000 cm−1 vary as a function of time, those graphs correspond to the left y scale. The transformation from oxy-Hb to met-Hb is illustrated by plotting the ratio 1589 cm−1/1640 cm−1 versus time. The time-dependent signals and ratios have been fitted to exponential functions. The ratio calculated from a spectrum taken from a met-Hb crystal is illustrated as a thick line at 1.1 (right y scale). Note that the values from the ratio from the last Raman recording and from the met-Hb crystal are almost identical. ![]() To investigate if the changes in spectra were due to thermal processes caused by light absorption, we performed estimates of the heat transport in the cell (see Sec. 2). The calculations indicate a temperature increase around 2.3°C at the laser focus for an incident power of 0.7 mW. Since RBCs normally have body temperature (∼37°C) and the measurements were performed at room temperature (∼20°C), a temperature rise of a few degrees should not harm the cells. Thus, we can rule out thermal degradation as the mechanism for the conversion of the hemoglobin state. The change of Hb state with time was fitted with an exponential function with a time constant k=0.012 s −1. In addition, concurrent changes in spectra revealed an increase of the fluorescent background. The increase in fluorescence could also be well fitted using a single exponential function, which gave a time constant kF=0.0074 s −1. We thus observed three laser-induced processes: 1. a decrease in Raman intensity, 2. a change in Raman spectra (oxy-Hb to met-Hb), and 3. an increase in fluorescence intensity. We cannot rule out the possibility that the decrease in Raman intensity is due to the change in oxidation state, because we do not know the absolute Raman cross sections of met-Hb and oxy-Hb. Another interesting possibility is that met-Hb is intrinsically fluorescent. The magnitudes of the rate constants for the change in Raman spectra and the fluorescence increase were almost the same, within experimental error, which indicates that this is possible. We have also observed strong fluorescence from commercially available crystalline met-Hb (Sigma), which further supports this scenario, although fluorescence from impurities cannot be ruled out in this case. The intrinsic fluorescence of Hb in various states has been well studied in the UV region, where the signal arises from tryptophan and tyrosine residues, since it might be used as a probe to study conformational changes in Hb and possibly other heme-containing proteins.18 19 However, little is known about the fluorescence of the heme in the visible. Early studies have shown that the fluorescence of these residues is strongly reduced in Hb, because of the efficient energy transfer from the amino acids residues to the heme.20 By replacing the heme by the corresponding metal-free porphyrin (Hb desFe ), fluorescence can be observed using visible excitation. The possibility of intrinsic fluorescence in the visible of unmodified met-Hb is interesting and needs further investigation. From these results it is clear that care should be taken to avoid photo-induced Hb chemistry. To prevent photo-induced effects, the irradiation power and the illumination time were decreased as much as could be tolerated from a S/N- and S/B-ratio point of view. Unfortunately, global Raman images of RBCs, with the majority of the Hb in the deoxy state, centered at the marker frequencies 1607 (deoxy), 1640 (oxy), and 2000 cm−1 (fluorescence background), were almost identical. The spectral resolution of the bandpass filter is ∼20 cm−1, therefore incorporation of neighboring bands cannot be ruled out in the 1640 cm−1 region, since there are C=C bands occurring at 1627 cm−1. A further problem we found concerning global Raman imaging was due to the increase of the fluorescence background with time. Therefore, Raman images recorded at the same spot but after different illumination times will differ even when taken at an off-peak spectral region. Obviously this hampers reliable background correction of a Raman image. We could not distinguish different Hb states in the same cell, if such a spatial distribution existed. Excitation with a different laser wavelength might reduce the fluorescent background. Recently, the characteristics of the Raman spectra of Hb from single erythrocytes using 632.8-nm excitations have been published,10 11 which is very promising for Raman imaging. In general, Raman imaging will give valuable information about the distribution of a compound in a sample, which is evident from further imaging experiments taken of the RBCs. Although we did not detect a spatial spectroscopic contrast within the RBCs, as noted before, we did observe a spatial contrast, primarily the doughnut morphology of the cell. Changes of the RBC membrane after adsorption to the different surfaces were observed in microscope images as well as in the global Raman images, as shown in Fig. 3. RBCs adsorbed to surface A, displayed in Figs. 3(a) to 3(c) exhibit fluctuating protrusions located in the doughnut ring directly after adsorption to the surface. These protrusions, which appear as “vibrating bubbles” in the cell membrane, are not seen when the RBCs are floating freely in the buffer solution. After a photon dose of ∼1.16⋅1019, the bubbles become static. The membrane appears to undergo photo-induced changes, a finding that needs further investigation. Cell membrane protrusions are also evident in the global Raman images, in which case they can easily be misinterpreted as a subcellular spectroscopic contrast within the cell. Indeed, Raman imaging involves some risk of misinterpretation of the results, because the recorded signal may be biased by variations in thickness across the sample layer.14 Figure 3(a) Brightfield image of RBCs adsorbed to surface A, (b) brightfield image of the RBCs seen in (c), which is a global Raman image taken at 1640 cm−1. The next row, (d) through (f) shows the same for surface B. The integration time of all global Raman images was 480 s with an irradiance of 450 W/cm2. The diameter of the RBCs is around 8 μm. ![]() Figures 3(d) to 3(f) show images of RBCs adsorbed to surface B. Flushing surface B with buffer showed that the RBCs remain fixed, thus we have much stronger adhesion strength to this surface. The RBCs appear to be much darker compared to the ones adsorbed to the commercial prep slide, and the doughnut shape of the cell is not visible in the same way as for surface A. The parts, which are light in Fig. 3(a), appear dark in Fig. 3(e), which indicates that the RBC has lost its doughnut shape. The global Raman image, centered at 1640 cm−1, [see Fig. 3(f)], reveals the same “inverted” distribution of light intensity as the brightfield image, which indicates that the majority of Hb is situated in the center of the cell and not at the edge. This confirms the loss of the native shape of RBCs adsorbed on surface B. Nevertheless, despite the changes in the RBC morphology, Hb within the cell remained functional when applying short integration times and low irradiance, i.e., 30 s and 6 kW/cm2, respectively. This is demonstrated by taking a series of Raman spectra during the oxygen uptake, and release, of a single RBC (see Fig. 4). The Hb state shifts from a deoxygenated state, after sodium dithionite has been added, to an oxygenated state on exposure to air. This process can be directly observed in Raman spectra as a shift of the ν10 band from 1640 to 1607 cm−1, and a shift of the ν19 band from 1589 to 1552 cm−1. The reversibility of the process proves that studying Hb dynamics in a single RBC is feasible. Note that the time course of the oxygenation cycle is limited by the diffusion of oxygen to the red blood cells after the depletion of oxygen by addition of sodium dithionite. Compared to the commercial poly-prep slide, this preparation method has the advantage that the sample can be flushed with new buffer, since the RBCs adsorb strongly to surface B. Thus, by being able to manipulate the environment of RBCs, the dynamics of Hb can be investigated in real time. Figure 4Series of spectra showing the oxygenation cycle of Hb within single functional erythrocytes. Integration time per spectrum is 30 s, with an irradiance of 6 kW/cm2; time between spectra is 20 s. Full lines indicate oxy-state markers while dashed lines indicate deoxy-state markers. Spectrum (a) is the characteristic oxy-spectrum. Between (a) and (b) sodium dithionite is added. (b) is a typical deoxy-spectrum that turns into the oxy spectrum (f) with time. ![]() 4.ConclusionsWe compared two different polylysine-coated glass slides regarding feasibility of Raman spectroscopy of functional red blood cells, namely a commercial slide (surface A) and an inhouse coated glass slide (surface B). We found two effects influencing Raman images and spectra of the RBCs. Changes of the cell membrane due to surface-induced effects could be observed immediately after adsorption of the cell to the surfaces, and were revealed in Raman as well as brightfield images. Photo-induced effects caused by the laser irradiation, e.g., the appearance of fluorescence intensity and a change in Hb state, were observed for both surfaces. An overview of the different Hb states that we have observed in this study is shown in Fig. 5. Since the fluorescence background hampers collection of Raman spectra, the best approach is to reduce the irradiance and the illumination time as much as possible while still getting good images or spectra. The surfaces showed different adsorption strengths. In the case of surface A, it was possible to wash away the cells by gently flushing the sample with buffer, whereas surface B showed much greater adhesion strength. The stronger adhesion allows for single-cell spectroscopy while adding different reagents or changing buffer. This is a great benefit and the method is promising. Figure 5Schematic illustration of the different states of Hb observed in this study, and how they were obtained. ![]() In the future, information on how Hb dynamics are influenced by the RBC response to different environmental stimuli, for instance exposure to different biomolecules like nitric oxide, can be obtained. Raman imaging might reveal how components within a cell rearrange on adsorption to a surface, which is most interesting when studying biocompatibility of different materials. We regard the method suitable for the study of Hb within single RBCs, since both dynamics of Hb and changes of the cell membrane can be investigated in real time. AcknowledgmentsWe acknowledge financial support from the Swedish Foundation for Strategic Research. We would also like to thank O¨rjan Hansson and Daniel Malmodin (Department of Biochemistry and Biophysics, Go¨teborgs University) and Fredrik Ho¨o¨k (Department of Applied Physics, Chalmers University of Technology) for valuable advice. REFERENCES
J. R. Pawloski
,
D. T. Hess
, and
J. S. Stamler
,
“Export by red blood cells of nitric oxide bioactivity,”
Nature (London) , 409 622
–626
(2001). Google Scholar
P. Rauenbuehler
,
K. A. Cordes
, and
J. M. Salhany
,
“Identification of the hemoglobin binding sites on the inner surface of the erythrocyte membrane,”
Biochim. Biophys. Acta , 692 361
–370
(1982). Google Scholar
H. Brunner
and
H. Sussner
,
“Resonance Raman scattering on haemoglobin,”
Biochim. Biophys. Acta , 310 21
–31
(1973). Google Scholar
T. G. Spiro
and
T. C. Strekas
,
“Resonance Raman scattering of heme proteins. Effect of oxidation and spin/state,”
J. Am. Chem. Soc. , 96
(2), 338
–345
(1974). Google Scholar
T. C. Strekas
and
T. G. Spiro
,
“Hemoglobin: Resonance Raman spectra,”
Biochim. Biophys. Acta , 263 830
–833
(1972). Google Scholar
S. Hoey
,
D. H. Brown
,
A. A. McConnell
,
W. E. Smith
,
M. Marabani
, and
R. D. Sturrock
,
“Resonance Raman spectroscopy of hemoglobin in intact cells: A probe of oxygen uptake by erythrocytes in rheumatoid Arthritis,”
J. Inorg. Biochem. , 34 189
–99
(1988). Google Scholar
C. W. Ong
,
Z. X. Shen
,
K. K. H. Ang
,
U. A. K. Kara
, and
S. H. Tang
,
“Resonance Raman micro-spectroscopy of normal erythrocytes and plasmodium berghei-infected erythrocytes,”
Appl. Spectrosc. , 53
(9), 1097
–1101
(1999). Google Scholar
B. R. Wood
,
B. Tait
, and
D. McNaughton
,
“Micro-Raman characterisation of the R to T state transition of haemoglobin within a single living erythrocyte,”
Biochim. Biophys. Acta , 1539 58
–70
(2001). Google Scholar
B. R. Wood
and
D. McNaughton
,
“Micro-Raman characterization of high- and low-spin heme moieties within single living wrythrocytes,”
Biopolymers Biospectroscopy , 67 259
–262
(2002). Google Scholar
C. Xie
,
M. A. Dinno
, and
Y. Li
,
“Near-infrared Raman spectroscopy of single optically trapped biological cells,”
Opt. Lett. , 27
(4), 249
–251
(2002). Google Scholar
G. J. Puppels
,
J. H. F. Olminkhof
,
G. M. J. Segers-Nolten
,
C. Otto
,
F. F. M. De Mul
, and
J. Greve
,
“Laser irradiation and Raman spectroscopy of single living cells and chromosomes: Sample degradation occurs with 514.5 nm but nor with 660 nm laser light,”
Exp. Cell Res. , 195 361
–367
(1991). Google Scholar
R. Salzer
,
G. Steiner
,
H. H. Mantsch
,
J. Mansfield
, and
E. N. Lewis
,
“Infrared and Raman imaging of biological and biomimetic samples,”
Fresenius J. Anal. Chem. , 366 712
–726
(2000). Google Scholar
P. J. Treado
,
I. W. Lewin
, and
E. N. Lewis
,
“High fidelity Raman imaging spectrometry: A rapid method using an acousto-optic tunable filter,”
Appl. Spectrosc. , 46
(8), 1211
–1216
(1992). Google Scholar
K. Ramser
,
E. J. Bjerneld
,
C. Fant
, and
M. Ka¨ll
,
“Raman imaging and spectroscopy of single functional erythrocytes: a feasibility study,”
Proc. SPIE , 4614 20
–27
(2002). Google Scholar
Z. Gryczynski
,
J. Lubkowsky
, and
E. Bucci
,
“Intrinsic fluorescense of hemoglobins and myoglobins,”
Methods Enzymol. , 278 538
–569
(1997). Google Scholar
R. E. Hirsch
,
R. S. Zukin
, and
R. L. Nagel
,
“Intrinsic fluorescence emission of intact oxy hemoglobins,”
Biochem. Biophys. Res. Commun. , 93
(2), 432
–439
(1980). Google Scholar
P. Sebban
,
M. Coppey
,
B. Alpert
,
L. Lindqvist
, and
D. M. Jameson
,
“Fluorescense properties of porphyrin-globin from human hemoglobin,”
Photochem. Photobiol. , 32 727
–731
(1980). Google Scholar
|
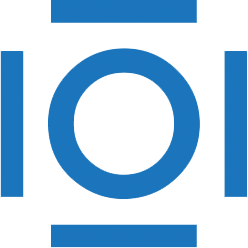
CITATIONS
Cited by 42 scholarly publications.
Raman spectroscopy
Luminescence
Raman scattering
Glasses
Adsorption
Blood
Oxygen